Synapses and protein molecules are involved in the formation of our memories. Synapses can be likened to “devices,” while protein molecules serve as the “components” of memories. In fact, these protein molecules are constantly regenerated and replaced within quite a short period of time. Despite this regeneration, the brain can retain memories for decades —— a truly astonishing example of the abstruse mysteries of life. It is thought that there might be some kind of mechanism between the synapses and the protein molecules. Accordingly, scientists say that looking at the actual site where memories are formed is essential in order to unravel the puzzle of what that mechanism might be.
Special Feature 1 – The Evolution in Visualization How can we retain memories? A look at the site of memory formation in the brain
composition by Rie Iizuka
illustration by Koji Kominato
I would like to shed light on the mechanisms supporting brain functions by observing molecular behavior.
Allow me to start by providing a simple explanation of the mechanism of memory, which is one of the brain’s major functions.
If you ask a 50-year-old, for example, what their earliest memory is, they are likely to say something like, “I remember walking along holding my mother’s hand when I was three or so.” Memories of this kind are stored in the cerebral cortex, via the hippocampus.
How has that person’s cerebral cortex changed since they were three years of age? If we were talking about a computer rather than a person, “remembering” means that the data is written on the memory, so it would probably have continued storing the same information without even needing to replace the hard disk over the previous 50 years. However, with people, it is a different situation.
Why are we able to continue retaining memories?
On average, the various protein molecules that normally function in the brain are replaced every 10 to 19 days, with some being replaced in as few as five days or so. This means that by the time the aforementioned 50-year-old has reached that age, not a single molecule remains from among the molecules that first recorded that memory when the individual was three. In other words, elements with a much shorter life span than the duration of the memory keep retaining that memory from half a century ago.
How is it possible to continue retaining memories even though the “components” (molecules) are constantly changing?
The devices involved in the formation of memories in the brain are structures called synapses (Figure 1). They are just 1 μm (1 thousandth of a millimeter) wide that form a junction between neurons, which transmit information. Presynaptic neurons release large quantities of the protein molecules glutamate and γ-Aminobutyric acid (GABA,) which function as neurotransmitters. These are caught by neurotransmitter receptors on postsynaptic neurons.
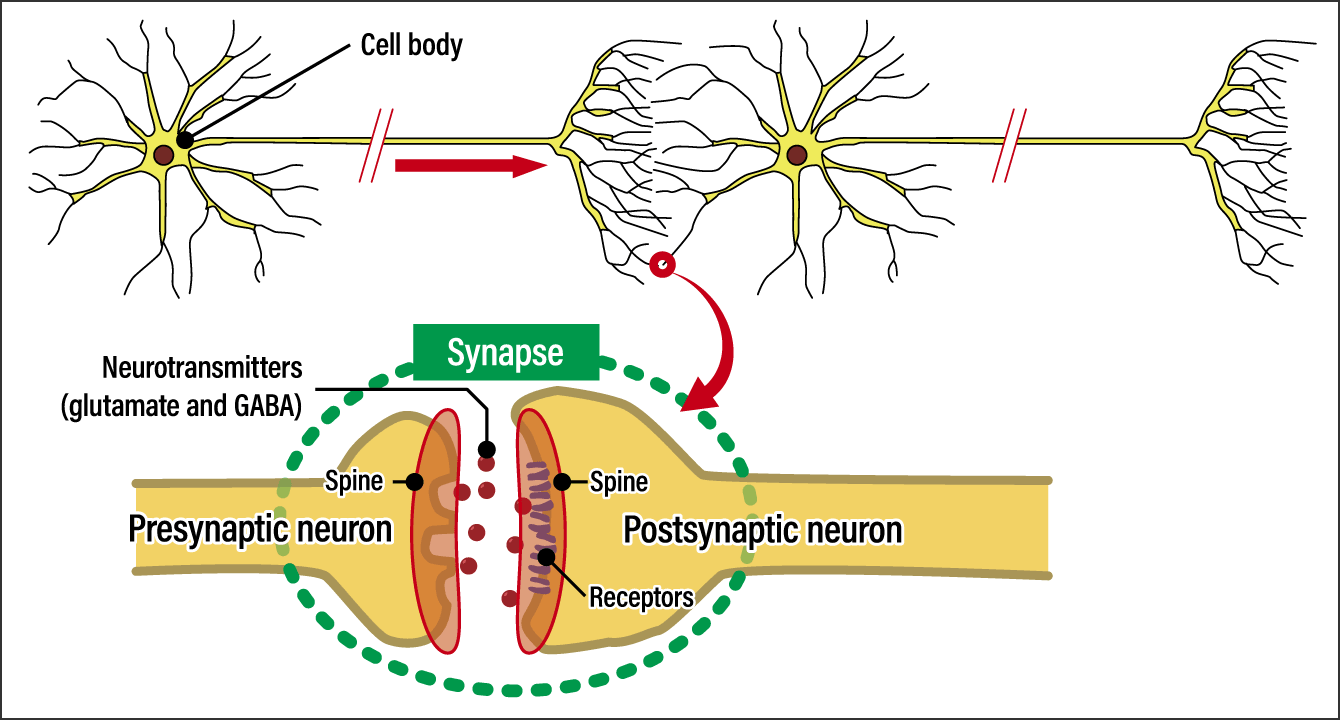
Figure 1. The structure of a synapseThis shows the spatial structure between the output side and input side of a neurotransmission signal. This spatial structure at the end of the long axons projecting from the cell body is called a synapse. The output side is called the presynaptic neuron, while the side with receptors is called the postsynaptic neuron.
If we think of a neurotransmitter released from a presynaptic neuron as a key, the receptor on the postsynaptic neuron could be likened to a door. If you use the key, the door attached to the cell will open and the positive ions Na+ and Ca2+ or negative ion Cl– in the extracellular fluid will rush into the next neuron. So, what actually happens when neural information is transmitted is that the next cell is filled with ions. The more doors there are in the postsynaptic neuron, the more positive ions will pass through them, resulting in powerful information transmission.
The stronger synapse function, the more stable memories are. More specifically, it means that the part of the synapse called the spine physically becomes larger, the number of receptors increases, and the quantity of neurotransmitters is higher.
In synapses, which are the site of memory formation, the reason why memories are retained even though the receptors and other raw materials are constantly being replaced is thought to be down to some kind of mechanism involved in the replacement of the components mdash;mdash; that is to say, the molecules. One can only marvel at the life system that enables us to continually retain memories and live our lives as the same person we were yesterday, even though none of the molecules present a month earlier remain.
Looking at the site where synapses and proteins actually operate
Accordingly, I decided to “look” at the site where memories are formed. In a world first, Dr. Antoine Triller of ENS in Paris observed the clustering of receptors in the cell membranes of postsynaptic neurons with the aid of immunofluorescence, an observation technique that involves attaching a fluorochrome to each individual receptor. The more receptors in the cluster, the higher the number of fluorochromes, so the cluster will look brighter. Observation under a microscope after applying another technique for making them easier to see revealed pronounced clustering in the postsynaptic membrane. This then gave rise to the question of why receptors are concentrated in the postsynaptic membrane, which is the margin of the cell.
More recently, researchers have focused on the molecular mechanisms that connect the small gaps between presynaptic and postsynaptic neurons. If this mechanism ruptures, neurotransmission does not take place. The collapse of this mechanism has actually been reported in cases of memory impairment and paralysis of the body caused by spinal cord injury, Alzheimer’s disease or cerebellar ataxia. Researchers in the laboratory of Professor Michisuke Yuzaki at Keio University have observed that introducing artificial molecules that strengthen connections between synapses into spinal cord injury model mice caused previously immobile hind legs to become mobile again.
The synaptic cell membrane is the place where proteins and receptors all clustered. However, these molecules are constantly flowing as the cell membrane is fluid (Figure 2). This means that even if the molecules cluster there temporarily, they cannot remain in that place indefinitely. In addition, as the life span of the molecules is a matter of days, there is a possibility that they will degenerate, so either way, they should progressively be replaced by new receptors.
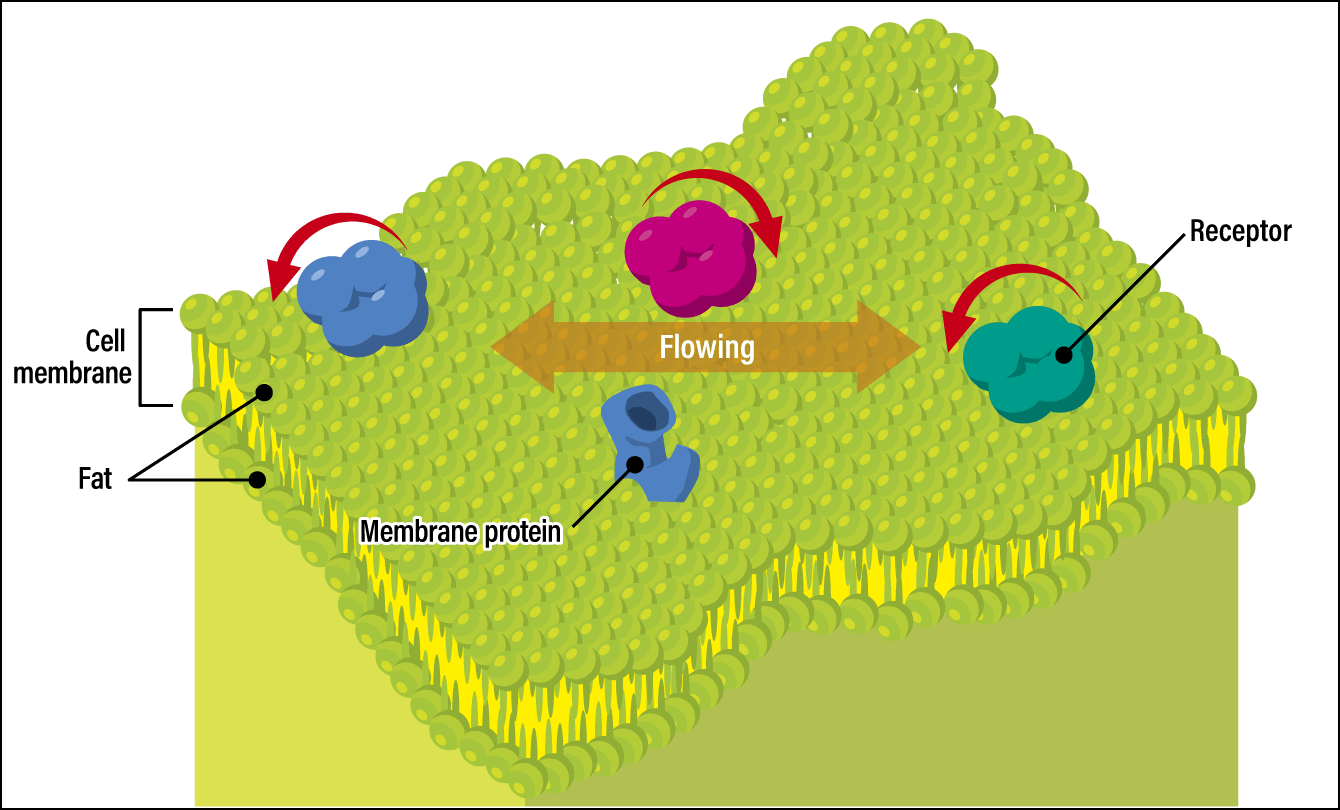
Figure 2. A cell membraneThe cell membrane structure features a dual layer of lipid molecules, with a hydrophilic group (molecules attracted to water) located on the outside, while a hydrophobic group (molecules attracted to lipids) faces inward. The upper surface of the membrane is constantly undulating, causing the receptors and membrane proteins to gradually move.
There are always around 50 synaptic receptors on the membrane surface which is very fluid, so, the receptors present are constantly coming and going. One could liken it to Shibuya Scramble Crossing in Tokyo, which is always crowded with people. There are lots of people there and it becomes even busier when some kind of event is going on. However, the people using the crossing are always different. The number of people in this scenario equates to the strength of the synapse. The important thing is where they gather. So why do molecules get trapped at this location? In an effort to unravel this question, I observed how molecules behave.
Attaching markers to molecules and recording their movements
Watching molecular behavior is something akin to the deductive process of the detective Sherlock Holmes. Holmes observes his assistant Dr. Watson and guesses what he is thinking about in that moment. He is able to do that because of information deduced from Watson’s behavior. Similarly, there is meaning to be found in the way molecules behave; if movement at one location slows down or becomes smoother, it indicates the presence of a condition that differs from the situation up to that point. This is why we observe the movement of molecules.
The observation technique is very simple: attaching markers to the molecules and recording their movements. When scientists first embarked on this research back in 2005, the use of quantum dots as markers must have been groundbreaking. Used in computer displays and the like, quantum dots are semiconductor crystals measuring less than 10 nm on each side. At the time, there were two principal methods of in vivo observation.
One involved staining the molecules with a fluorescent dye for observation, but the amount of time for which they could be observed back then was no more than a few seconds. Consequently, it was virtually impossible to observe the course of movements of a single molecule entering and leaving again. The other technique involved attaching metal tags, but it was unsuitable for this research, because they could not get into the tiny gaps between the cell membranes. Accordingly, as quantum dots are effective for a relatively long time —— a whole minute —— and are also physically small, they proved to be a very user-friendly tool for observing molecular behavior.
A large number of molecules called scaffold proteins, which are capable of momentarily binding to receptors, gathered in the area where the receptors were clustered. The movement of the receptors in this area slowed down and, as a result, their concentration increased. They had been forced to slow down by a kind of traffic jam.
It seems unsurprising that receptors should cluster there, since that is where the synapse is located, but after considering the fact that the cell membrane is fluid and mobile, it was found that the receptors keep moving, but slow down as needed. If the receptors were to bind tightly to a synapse, the synapse would actually cease to function, as the receptors have a life span of several days. Thus, part of the mechanism that keeps an individual alive during a constant process of maintaining the body was revealed.
In addition, Dr. Fumihiro Niwa, an assistant professor at Jichi Medical University, has reported that calcium, which is frequently used as a signal within cells, inhibits the movement of receptors. Mice lacking intracellular signals suffered from epilepsy and lived for only 20 days or so. The research team also discovered that the mobility of one type of receptor are increased in these mice. As a result, the signals that enable the brain to function normally are not transmitted and cause malfunction.
Thus, the behavior of a single molecule that causes a disease is being unraveled by observing the movement of synapses and molecules.
Offering a new perspective on the life sciences
Another study aimed at shedding light on a brain mechanism via observation is research into astrocytes, a type of glial cell, which was conducted by Dr. Misa Arizono, a program-specific associate professor at Kyoto University’s Hakubi Center for Advanced Research.
Astrocytes control the dilation and contraction of cerebral blood vessels and the supply of nutrition to the brain, and sometimes directly control synaptic transmission, thereby helping to complement the workings of neurons. Consisting of a cell body surrounded by projections known as processes, they are shaped like stars and form complex shapes as they cover and inhibit synapses or cling tightly to the surface of blood vessels.
Within astrocytes, calcium signaling predominantly takes place in the processes, for some reason. When a stimulus that increases calcium signaling is applied to cultured cells, the calcium level unquestionably increases from the processes in almost 100% of cells. In other words, the processes have a higher calcium sensitivity.
To investigate this mechanism, the research team first looked at where in astrocytes the calcium receptors were located. As the cells are linked and the membranes are fluid, the receptors should be able to move anywhere, but they were only observed in any number at the margins of the processes, with hardly any in the cell body. The team then carried out single-molecule imaging based on these results. This involved binding quantum dots to the receptors responsible for calcium signaling in a single molecule and observing them. Observation was possible for around 10 minutes, but not a single molecule ultimately entered the cell body.
They demonstrated brisk movement at the borders of the processes, which means they are able to move under the conditions of the experiment. Overlays of the range within which the molecules moved over the course of a 10-minute period showed a distinct area empty of molecules, where molecules had not entered the cell body. Even in the processes, there are areas that the molecules enter and areas that they do not; looking at the molecules’ movement, the research team discovered that compartmentalization takes place inside the cell membrane (Figure 3).
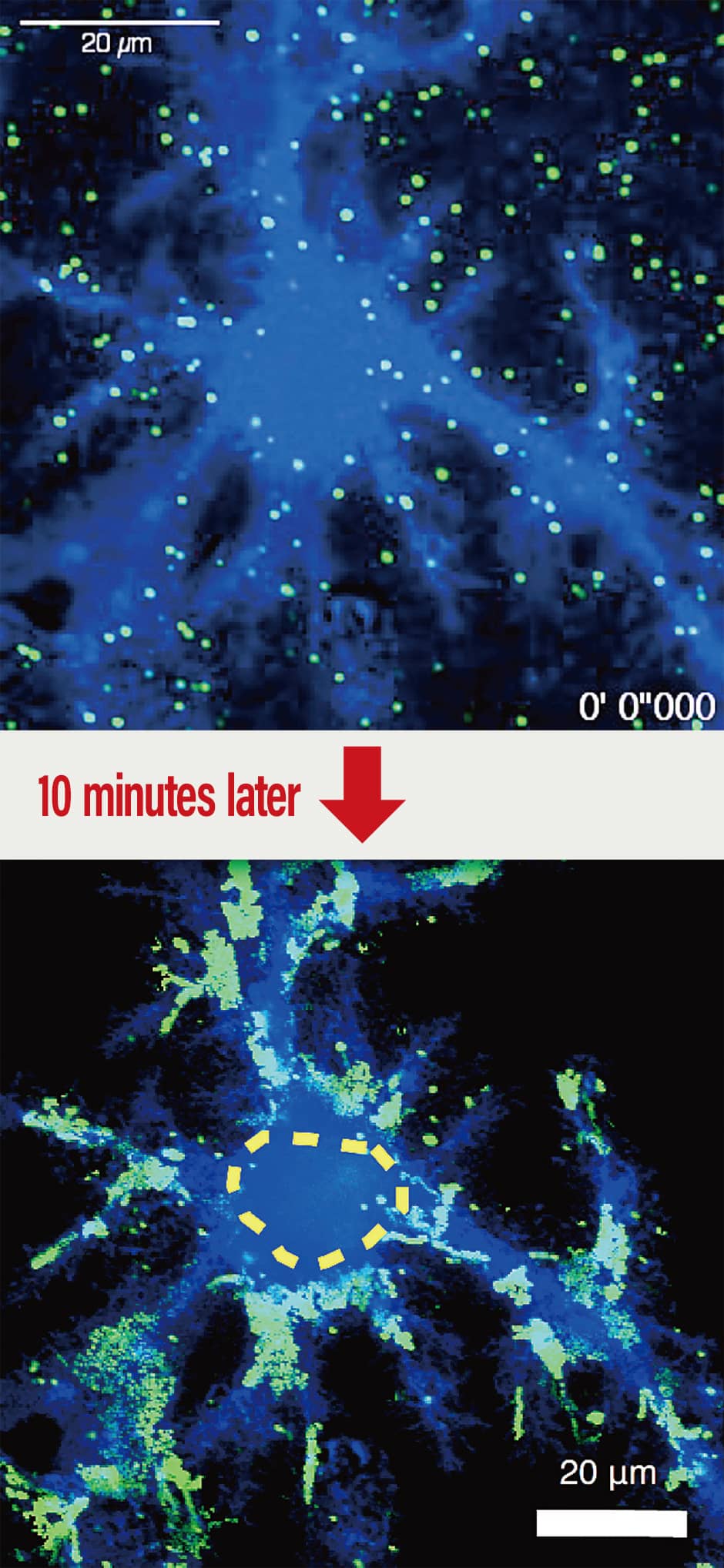
Figure 3. Example of compartmentalization in an astrocyte cell membraneThe spots of green light are metabotropic glutamate receptors. Glutamate is one of the substances that triggers calcium signaling in astrocytes. When researchers used quantum dots to label (in green) the metabotropic glutamate receptors that receive glutamate and track their movement, they did not see any green light in the cell body, which is indicated by the yellow dotted line. This demonstrated that the movement of metabotropic glutamate receptors is confined to astrocytic processes alone.
Furthermore, it was found that overexpression of those molecules in the cell or inhibition of their interaction with other proteins in the cell frequently causes calcium signaling to occur not only in the processes, but also in the cell body. It is conceivable that some kind of mechanism in the processes that acts as a barrier to calcium signaling had broken down. This then causes defects in the control of synapses and blood vessels, among others. In fact, the barriers in these processes are already known to have failed in the brains of Alzheimer’s patients.
Observation has revealed that molecular movement is not uniform, even within a single astrocyte. It would appear that compartmentalization takes place, as there is probably some information that ought to spread throughout the brain, while other information can be limited to an enclosed area. I would like to gradually shed light on the mechanisms supporting the control of these compartments.
If you were to ask me why we should focus on movement now that we can readily study omics, which involves comprehensive investigation of the molecules in a body, I would say that it is because it is taking place at the site where life actually happens. In which cell locations things are happening and how the molecules there behave is of key significance to biological activity. The only way to find out is by actually looking at the cells. I believe that looking at molecular behavior will offer us yet more new perspectives on the life sciences.