Jellyfish, fireflies, and other organisms that emit light have their own unique luminescence mechanism. The first to be discovered was the protein that makes the jellyfish Aequorea victoria glow. Subsequently, this fluorescent protein was applied in the development of in vivo fluorescence imaging, enabling cells and tissues to be observed in real time while still alive. Life sciences research progressed substantially as a result. High-precision visualization is now possible, thanks to the development of imaging techniques that leverage the luminescence mechanisms of various organisms. These techniques are contributing to a multitude of areas, including the kinetic analysis of cancer cells.
Special Feature 1 – The Evolution in Visualization Bioluminescence imaging and real-time cell observation
composition by Yumi Ohuchi
Earth is home to a number of creatures that emit light, such as jellyfish and fireflies. Dr. Osamu Shimomura, who conducted research that shed light on these luminescence mechanisms, published a paper in 1962 after discovering green fluorescent protein (GFP) in the jellyfish Aequorea victoria. A few decades later, Dr. Martin Chalfie implanted the GFP gene into cells from the nematode Caenorhabditis elegans and succeeded in expressing GFP outside Aequorea victoria, causing the organism’s cells to light up.
Dr. Roger Y. Tsien produced GFP variants, developing fluorescent proteins in a range of colors besides green. All these research achievements laid the foundations that have given us the ability to observe living cells and tissues in real time, using fluorescence as a marker (fluorescent label). Called in vivo fluorescence imaging, this technology has made a substantial contribution to advancing life sciences research. The outstanding achievements of these three researchers resulted in their being awarded the Nobel Prize in Chemistry in 2008.
Different ways to make organisms glow
So, how does the phenomenon of fluorescence actually occur in the first place? Normally, when a molecule is exposed to light, it absorbs the light and instantly discharges that energy in a form such as heat, vibration or light as it seeks to return to its original stable energy state. Fluorescent proteins glow because they readily emit fluorescence in this energy discharge process. Accordingly, in vivo fluorescence imaging requires a fluorescence microscope, which exposes the sample to light and observes the fluorescence it emits.
In fact, there are many other fluorescent substances —— substances that absorb light and emit fluorescence —— besides proteins. Fluorescent small organic molecules such as fluorescein, which is used in fluorescent bath salts, are one type found in everyday settings. In addition, some pharmaceutical substances glow, including the anti-cancer drug Adriamycin and indocyanine green (ICG), which is used in liver function tests. These substances, too, can be used in living organisms as fluorochromes, and have advantages that fluorescent proteins do not. Fluorescent proteins cannot be used in humans because the gene needs to be inserted into living cells. However, fluorochromes can be injected into humans and used for real-time observation. Fluorescein angiography and ICG angiography are already used in the field of ophthalmology, while other clinical applications are progressively being developed, such as in the realm of surgery, where ICG is used for determining the extent of resection and for fluorescent lymphangiography.
Some organisms glow via a mechanism other than that of fluorescent substances. For example, while the Japanese kanji character for firefly is the first character in the Japanese word for fluorescence, which translates literally as “firefly light,” the glow emitted by fireflies does not come from a fluorescent protein. This glow is actually produced by a chemical reaction between the enzyme luciferase and the light-emitting compound luciferin, which are both found in fireflies. As these substances glow without being exposed to light, they can be rendered visible by means of imaging with a highly sensitive camera in a dark place; bioluminescence imaging using this reaction has also become a useful tool for in vivo observation.
In our laboratory, we conduct both basic and applied medical research that leverages the features of each of these imaging technologies. One area on which our research has focused for many years is cancer. I myself am a cancer researcher and I would grind up or fix excised cancer cells and tissue and observe them. However, this does not allow us to view the movement of cancer within the body, nor can we observe the process within a single individual. Ever since those days, I have been most interested in how cancer actually moves within the body, and that was the catalyst for my research into imaging of this kind.
Analysis of cancer cell dynamics
Metastasis is a particular problem when it comes to cancer. These days, we can use surgical procedures to remove cancer if it is confined to a particular part of the body, but treating metastatic cancer is still very difficult. I believe that shedding light on the mechanisms of metastasis is vital.
One form of cancer metastasis is hematogenous metastasis, in which cancer cells travel in the bloodstream. Accordingly, we conducted an experiment in which we inserted fluorescent protein genes into human cancer cells and transplanted them into mouse blood vessels. We then observed that the cancer cells traveled within the blood vessels and exited them via the vascular wall (Figure 1). A key advantage of in vivo fluorescence imaging is that it gives us this ability to zoom in from the macro to the micro world, enabling us to conduct detailed analysis of the dynamics of a diverse array of cancer cells.
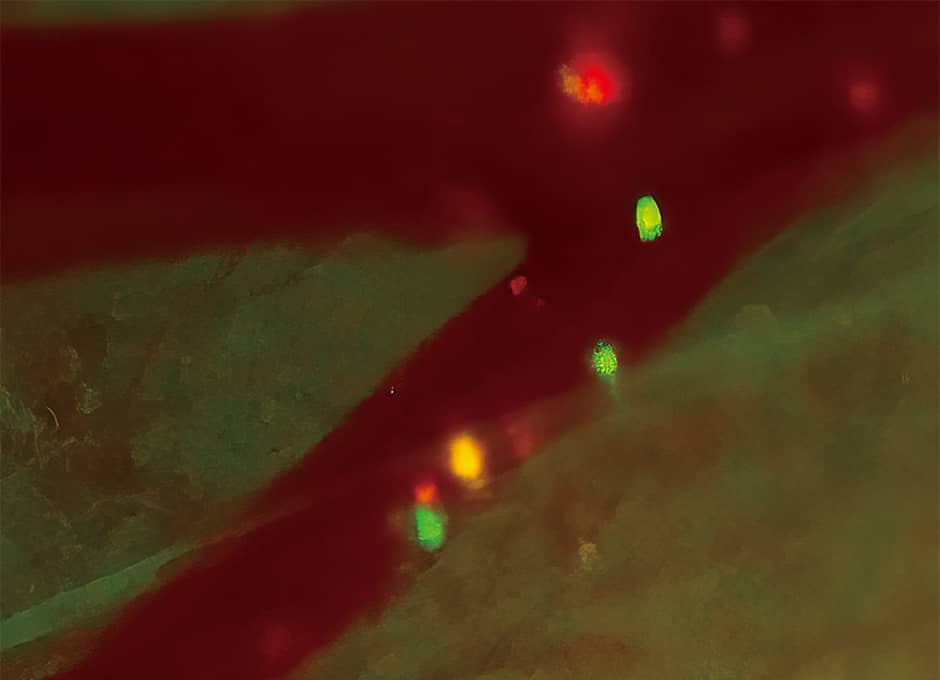
Figure 1. Movement of cancer cells within a blood vesselCancer cells that express a fluorescent protein traveling within a blood vessel. The spots of green light are cancer cells. (Experiment using a technique called the skin-flap method)
In addition, cancers form new blood vessels to travel throughout the body. Our experiments combining in vivo fluorescence imaging with bioluminescence imaging showed both this angiogenesis process and cancer cell dynamics. This technique has been applied to evaluate angiogenesis inhibitors, a type of anticancer drug.
Cancer may also metastasize into the lymph nodes via lymphatic vessels. Introducing different types of fluorochromes into the lymphatic vessels and blood vessels of mice into which cancer cells made to express fluorescent protein have been transplanted makes it possible to render the cells and vessels in separate colors. This allows us to simultaneously observe how blood vessels, lymphatic vessels, and lymph nodes are involved in the process of cancer metastasis.
We know that certain cancers have a tendency to metastasize into specific parts of the body, with breast cancer prone to metastasizing into the bones, while colon cancer often metastasizes into the liver. We have actually introduced the luciferase gene into human breast cancer cells, injected those cells into a mouse’s heart, and used bioluminescence imaging to observe the process of metastasis after administering luciferin. Immediately afterward, cancer cells that glowed bluish white were present throughout the body, but hardly any were visible once a week had passed. This is because cancer cells undergo cell death if they cannot find a stable foothold. However, three weeks later, we found cancer cells proliferating in the bones, providing visual verification of the tendency of breast cancer toward bone metastasis (Figure 2).
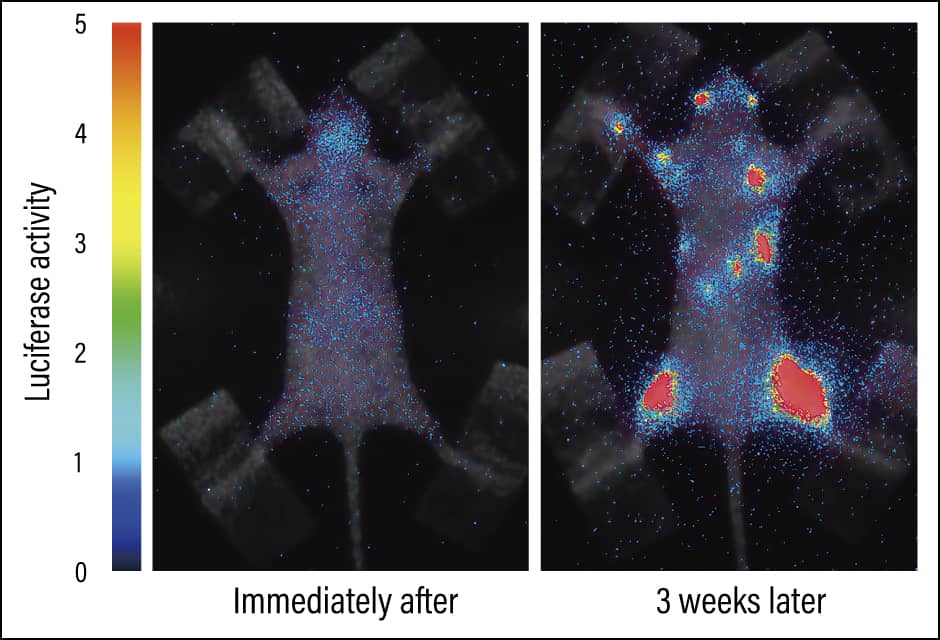
Figure 2. Bone metastasis of cancerBioluminescence imaging in a bone metastasis model. The areas that are glowing are cancer cells, and one can see that they have metastasized into the bones after three weeks. The light changes from blue to red, with red indicating a large number of cancer cells.
Thus, bioluminescence imaging has the advantage of being highly sensitive and making it possible to gain a broad-brush understanding of general changes. In vivo fluorescence imaging, on the other hand, not only has the aforementioned merit of being able to focus on finer areas, but also allows us to observe various functions if we use further genetic modification to adjust fluorescent proteins.
Understanding intracellular phenomena
The fluorescent ubiquitination-based cell cycle indicator (Fucci) developed by Dr. Atsushi Miyawaki of RIKEN is a system enabling the process of cell division and multiplication to be visualized in phases. More specifically, when the genes for red and green fluorescent proteins are bound to the genes for two types of protein present at specific phases in the cell cycle, and those two types of genes are introduced into cells, it is possible to observe the different phases from the different colors, with cell nuclei in the G1 or G0 phase of the cell cycle colored red, and cell nuclei in the S/G2/M phases colored green. G1 is the phase in which the cell is not multiplying, while the G0 phase is the state of quiescence. S is the DNA replication phase, G2 is the preparatory phase for cell division, and M is the phase in which cell division actually occurs.
In collaborative research with Dr. Miyawaki, we introduced Fucci into virtually normal cultured cells (normal cells) and cultured cells derived from a human cancer (cancer cells), then cultured them on petri dishes and investigated the differences between them. We found that the normal cells multiplied to some extent before reaching a point at which they no longer multiplied, with the nuclei of all the cells showing up red (indicating G1 or G0 phase). This is because the cells were grown on dishes and once they became densely packed, contact inhibition of cell proliferation occurred, curbing excessive proliferation. However, cancer cells continue to increase, building up even after space has run out. This abnormal proliferation is the distinctive nature of cancers. Using Fucci we can understand not only this proliferation, but also whether a cell is in a state of growth arrest or quiescence, or is in an active phase (Figure 3).
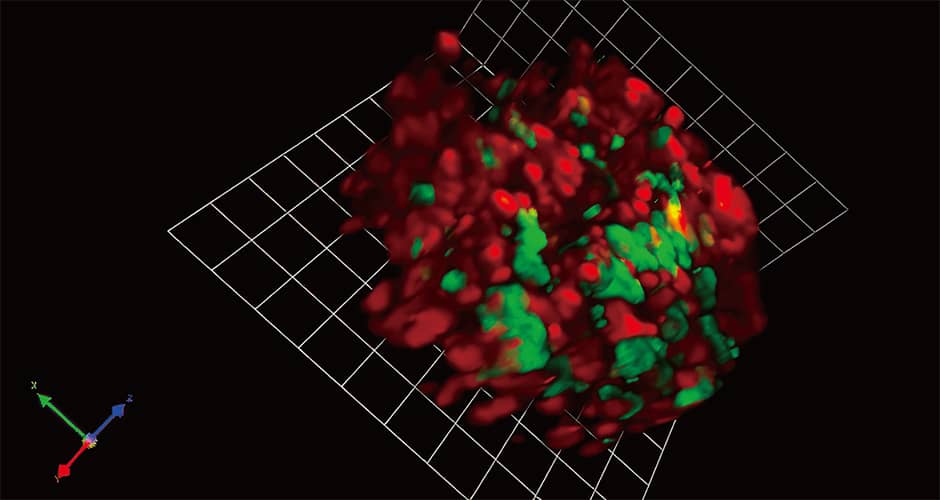
Figure 3. 3D fluorescence imaging using FucciBreast cancer cells that have metastasized into the bones. The red light indicates the G1 or G0 phase (in which cells are not proliferating), while the green light indicates the S/G2/M phases (in which they are dividing). Genetic modification of fluorescent proteins makes it possible to see with our own eyes information that was previously unknown.
We also succeeded in causing bone metastasis by transplanting into mice cancer cells into which we had inserted Fucci. In the bone metastasis lesions, almost all of the cells were in G1 or G0 phase to start with, but by the terminal stage, cells in G1 or G0 phase were mixed with active phase cells. When we then administered a cytotoxic anticancer drug, only cells in G1 or G0 phase survived. Cytotoxic anticancer drugs work by inhibiting cell division and multiplication, so one might say that this result is only to be expected, but this experiment provided a visible demonstration that administering such drugs outside the active phase is not effective.
In addition, it would appear that cancer cells in a state of quiescence are involved in cancer recurrence. We discovered that it is important for treatment approaches to target cancer cells in the G1 or G0 phase. That is to say, the two conceivable approaches are to either ensure the cells do not emerge from their state of quiescence, or to wake them from their hibernation and then hit them with anticancer drugs or radiotherapy. Currently, we are undertaking research using Fucci that aims to identify the trigger that wakes the cells from their slumber.
In addition, in collaborative research with Okayama University, we have developed a fluorescent protein that glows red when cancer cells undergo epithelial-mesenchymal transition (EMT). EMT is a metastatic process in which, to put it in simple terms, neatly aligned epithelial cells undergo a transformation into mesenchymal cells, which have foot-like projections, and develop the ability to move. Thus, in vivo fluorescence imaging has also given us the ability to gain an understanding of intracellular phenomena that hitherto could not be seen. EMT is thought to be one cause of treatment resistance in cancer; with the aid of this imaging technique, work is progressing on efforts to shed light on the mechanisms of cancer drug resistance and to develop inhibitory agents.
So, as described above, in vivo fluorescence and bioluminescence imaging are useful tools for unraveling cancer mechanisms, evaluating the effectiveness of therapeutic drugs, and developing new treatments. In terms of prospects for the future, light-based technologies could conceivably be applied to cancer surgery. The development of optical biopsy, which enables cancer to be diagnosed without excising any tissue, is already progressing. In addition, for some cancers, Japan’s medical insurance system covers photodynamic therapy, such as photoimmunotherapy, which involves attaching a substance that reacts to light to cancer cells and exposing them to a laser beam to destroy the cancer.
For example, multiple colors are used to light up blood vessels and tissues to aid in navigating the operative field, and if the optical biopsy shows a tumor to be malignant, the tumor will be excised while using light to check its extent. If photodynamic therapy is then used to remove any remaining cancer that could not be cut out, it will be possible to deal with the cancer in one fell swoop. Light also has the advantage that, unlike radiation, it imposes little burden on the body.
Microscopes and digital image processing systems are also crucial
In addition to technologies that make cells and tissues glow, microscopes and digital image processing systems for observing them are also very important. The members of our laboratory include individuals with backgrounds in science and engineering, and mathematical physics, and we have worked with optical instrument manufacturers to develop new microscopes.
One such device is a fluorescence microscope called a two-photon excitation microscope, which enables us to view deep tissue unable to be seen with conventional fluorescence microscopes. For example, when using in vivo fluorescence imaging to observe neurons in mouse brains, existing microscopes could only penetrate to a depth of a few hundred microns, meaning that one could not see much more than the surface of the cerebral neocortex, but using a two-photon excitation microscope makes it possible to penetrate as far as pyramidal cell bodies. Furthermore, using a special two-photon excitation microscope fitted with a new laser that we developed makes it possible to see as far in as the hippocampal cells (Figure 4). In addition, applying the two-photon excitation to a special kind of microscope called light-sheet microscope enables three-dimensional (3D) images to be captured at high speed and over a long period, thereby facilitating what could be described as 4D cell observation. Using this microscope, we succeeded in imaging the embryogenesis process of medaka fish (Oryzias latipes) over the course of three days, capturing the entire body at the cellular level in vivo.
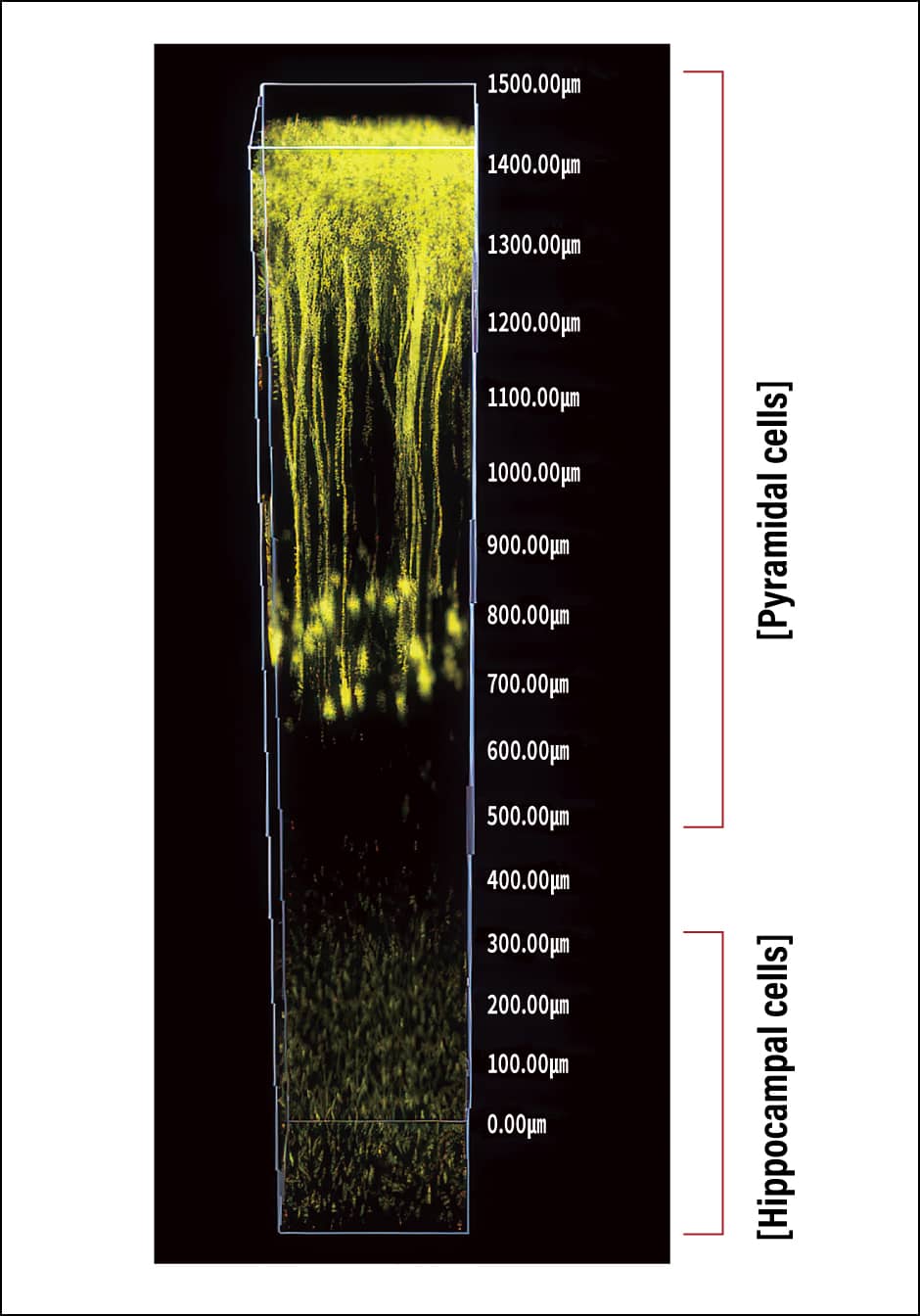
Figure 4. 3D fluorescence imaging using a two-photon excitation microscopeIt has become possible to see not only the pyramidal cells of the cerebral neocortex, but also hippocampal cells, which could not be viewed with a conventional fluorescence microscope.
Our laboratory has more than 10 other microscopes that we use for specific purposes. Improving microscopes of this kind takes a considerable amount of money, as well as technical skill, so these are not the kind of devices available to every research institution. Accordingly, our laboratory has joined a government research support program called Advanced Bioimaging Support (ABiS) and is supporting researchers nationwide who require imaging technology. This means that our laboratory attracts a variety of researchers from across Japan, thereby broadening the scope of our research. For example, in a research project conducted with Professor Toshiro Sato of Keio University, we are developing a special microscope for imaging organoids, which function like miniature organs. In the realm of skin diseases, we are working with dermatologists on the use of 3D fluorescence imaging to shed light on the pathology of palmoplantar pustulosis —— a disease whose precise cause is unknown and which is hard to treat —— and develop new diagnostic techniques. We are also working with breast surgeons on research into AI-based image analysis and automatic diagnosis.
Growing importance is being attached to real-world, real-time evidence; for example, video assistant refereeing has recently been introduced in the world of sports. The same applies to biological phenomena; the concept behind our research is that what we observe in real time becomes the first evidence of a phenomenon. Seeing is believing. More than half a century has passed since Dr. Shimomura discovered GFP, but in vivo observation has not yet become mainstream in molecular biology research. Going forward, I want to continue my quest to use optical imaging to shed light on biological phenomena and pathology, gathering together knowledge not only from the fields of molecular biology and medical science, but also from a diverse array of other realms, including optics, engineering, physics, and mathematical sciences.